Environmental Management of the Waitomo Glowworm Cave
Effects of Visitors and Ventilation on Carbon Dioxide Concentrations
School of Environment, The University of Auckland, New Zealand 2
Email: 1 gillies.matthew@gmail.com, 2 c.defreitas@auckland.ac.nz
Abstract
Environmental management of the Waitomo Glowworm Cave aims to mitigate the potential conflict between presenting the cave to visitors and protecting it. In the case of the cave’s microclimatic environment, it aims, firstly, to control rise in carbon dioxide (CO2) levels above a certain threshold, and secondly, to keep evaporation rates low so that cave drying is minimised. Reduced ventilation combined with CO2 from visitor respiration can lead to elevated CO2 levels in the cave. The CO2 combines with moisture in the cave to form carbonic acid that corrodes calcite features of the cave. This damage is essentially irreversible. High ventilation rates during cool external conditions can lead to cave drying. Managing visitor numbers and airflow through the cave can regulate both of these. Earlier work has shown that three ventilation regimes exist; namely, downflow, upflow, and neutral, which are determined by cave-to-outside air temperature gradient (Td). Cave ventilation can be regulated by a door at the cave’s upper entrance which, when closed, seals the entrance and restricts airflow though the cave. Ineffective management of cave ventilation and visitor numbers can affect cave microclimate thereby compromising the glowworms’ habitat. This study examines the extent to which cave management effectively minimises both cave drying and rising cave air CO2 concentrations in the cave. Four detailed monitoring experiments were carried out inside the cave over a 10-month period. The results of these experiments together with a statistical analysis of a five-year data archive show the extent to which visitor numbers and cave ventilation affect CO2 concentration in the cave. Overall, the results show: cave air CO2 levels are moderately related to visitor numbers; cave air CO2 levels are not related to Td; and cave drying is not related to Td. From this it may be concluded that cave management practices through ventilation and visitor control is effective.
Introduction
The cave that is the focus of this research is the Waitomo Glowworm Cave, an icon of New Zealand’s North Island tourist experience and one of the most heavily used tourist caves in the world in which cave fauna is the main attraction. Experience has shown that exceptional environments such as the Glowworm Cave must be carefully managed when used for tourism or recreational purposes. If the stability of the natural cave system is disturbed, deterioration of the cave can occur. Cave microclimate plays an important role in sustainable management of the cave because of its small size, the presence of speleothem features and climate sensitive fauna on which tourism relies (de Freitas, 2010). The cave’s microclimate is a complex function of many variables, such as cave rock temperature, moisture availability and air exchange with the outside. Airflow, however, is the main control.
The opposing pressures of presenting and protecting the cave require a balance to be met. In order to establish an acceptable equilibrium between the two demands, a sound understanding of the cave environment and processes operating within it is necessary. The tension between these two interests, namely financial and environmental, has been described by O’Brien and Watson (1977) as the ‘paradox of conservation’.
Description of the Glowworm Cave
The Glowworm Cave is located in the village of Waitomo near the centre of the North Island of New Zealand, about 160 km southeast of New Zealand’s largest city Auckland. The cave is positioned in a ridge of Oligocene limestone underlain by a Mesozoic greywacke basement. Its two entrances are separated about 14 m vertically apart and linked by approximately 1300 m of interconnected chambers and passageways (Figure 1). The cave can be separated into two distinct levels; the upper level, comprised of the Main Passage, the Blanket Chamber and the Organ Loft; and the lower level, comprised of the Banquet Chamber, the Cathedral, the Demonstration Chamber, and the Glowworm Grotto. The Waitomo Stream flows through the lower entrance into the Grotto, sumps for a short distance, and then emerges in the Demonstration Chamber. The stream sumps again near the end of the Demonstration Chamber before resurging outside the cave (de Freitas et al., 1982).
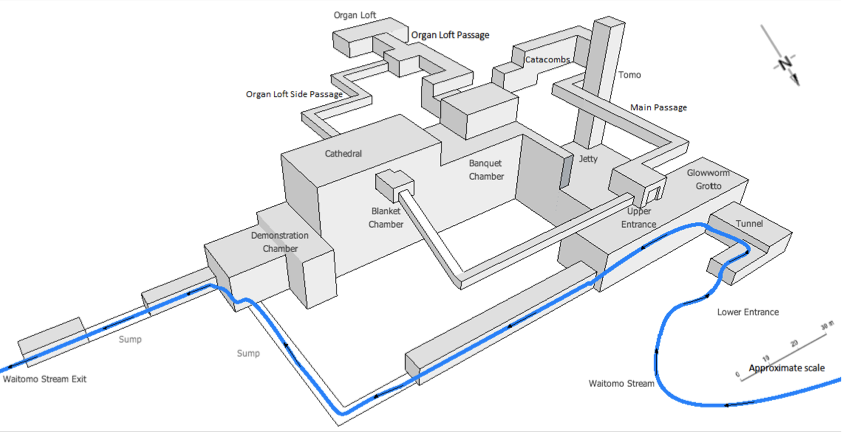
Figure 1: Schematic diagram of the Glowworm Cave at Waitomo, indicating the location of monitoring sites (after de Freitas et al., 1982: 384).
Since the Glowworm Cave is a two-entranced cave, air exchange with the outside takes place via efficient ‘chimney effect’ airflow downward and upward through the cave (Figure 2). Downflow occurs when outside air temperature is higher than that in the cave, as the relatively cool, dense cave air drains out. Upflow occurs when the cave air is warmer than that outside and the buoyant air is driven upwards by convection. Up-flow conditions lead to high evaporation rates in the cave and cave drying, both of which are damaging to the cave’s ecosystem. In transitional conditions, where the cave-to-outside-air temperature difference is small, there is little or no air exchange.
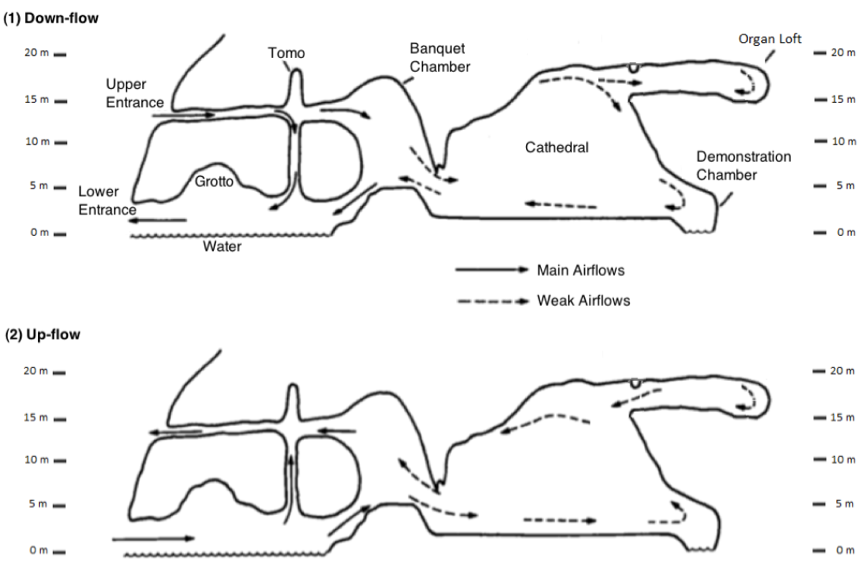
Figure 2: Cross-sectional view of the two thermally induced cave airflow regimes: (1) downflow when outside air temperature is higher than in the cave; and (2) upflow when the outside temperature is lower than in the cave (after de Freitas et al., 1982: 391).
Human use of the cave along with external influences can have a diverse range of impacts (Figure 3). Human use can affect ventilation through modification of cave entrances. Under certain conditions, high rates of air exchange lead to detrimental changes within the cave. Excessive evaporation and desiccation of the cave, as well as changes of temperature in the cave compromise the glowworms’ habitat (de Freitas, 2010). On the other hand, ventilation of the cave prevents the build-up of carbon dioxide (CO2). Elevated CO2 levels in cave air from visitor respiration combines with moisture in the cave to form carbonic acid that corrodes calcite features of the cave. Cave management aims to mitigate the potential conflict that exists between the dual requirements of presenting the cave and protecting it. The Glowworm Cave is operated under licence issued by the New Zealand Government’s Department of Conservation. The licence stipulates that the cave microclimate should be carefully monitored and maintained as to avert damage. The first condition is that CO2 levels should not be allowed to rise above 2,400 ppm. The second is that cave drying should be minimised during upflow. Strong, prolonged upflow can cause evaporation in the cave than would otherwise occur. Glowworms, which are positioned in the cave rock-cave air boundary layer, suffer as they act as a wick in the vapour transfer process.
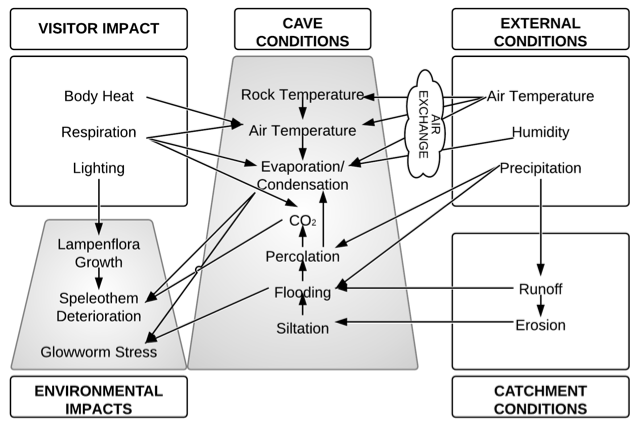
Figure 3: Key factors and processes affecting the Glowworm Cave (after de Freitas, 2010: 482).
The CO2 concentration in the cave air depends on the size and frequency of tour groups and the length of time they spend in the cave, on the one hand, and the strength of cave ventilation on the other. The strength of cave ventilation is a function of cave-to-outside-air temperature gradient (Td). In the case of the Glowworm Cave, ventilation is controlled by opening or closing an airtight door at the cave’s upper entrance as required. This way cave microclimate can be managed, except at times when Td is at or near zero. In practice, cave management is based on the fine balancing of tourist numbers and tour frequency with cave ventilation to minimise both evaporation and the rise of CO2 concentration in the cave air. In light of this, the research here examines the extent to which cave management effectively minimises both cave drying and rising cave air CO2 concentrations. Hence the aims of this study are, firstly, to assess spatial and temporal differences in ventilation and CO2 build-up in the cave and rates of evaporation within the cave, and secondly, to evaluate how effectively cave drying and CO2 are being regulated via active management.
Method
To achieve the stated aims of the research, two sets of data are used. Each is collected via automated monitoring systems and they are, hereafter, referred to as the: ‘long-term data’ and ‘experimental field data’.
The long-term data, which are used for the statistical analysis, is comprised of five years (2008-2012) visitor numbers, microclimate, and CO2 data. The data is taken from the Automatic Environmental Monitoring System (AEMS) that is permanently installed within the cave. Air temperature and CO2 measurements are made at 30-minute intervals and stored on Campbell Scientific CR10X data loggers. Measurements of cave air temperature (dry-bulb) are made with a Campbell Scientific 107B fan-ventilated thermistor located in the cave’s interior at the Banquet Chamber monitoring site (Figure 4). Another dry-bulb thermistor is located outside the cave nearby the Upper Entrance. Cave air CO2 concentration is measured using Vaisala GMP222 sensors located at the Cathedral and Organ Loft (Figure 4). Visitor data are comprised of totals of the number of visitors inside the cave at any given time, taken from cave tour-group admission sales logbooks.
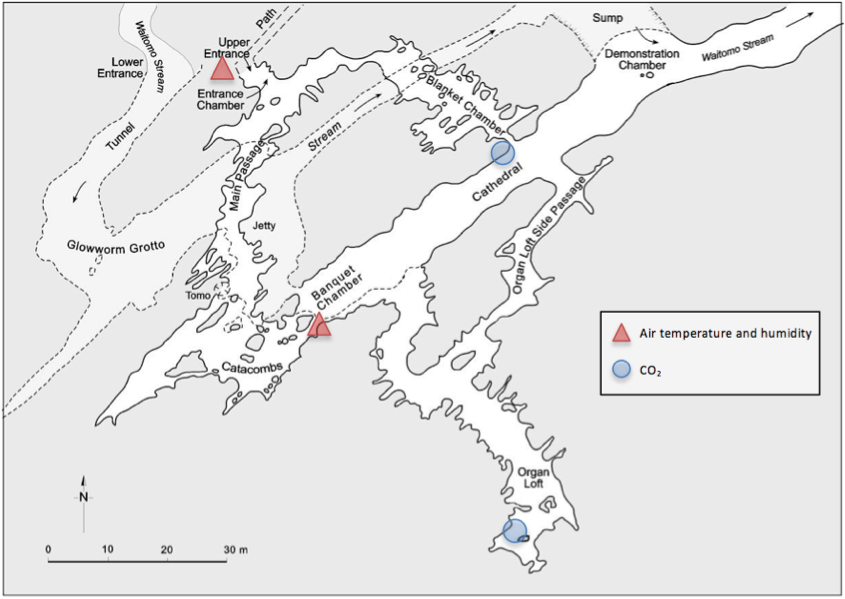
Figure 4: AEMS sensor monitoring sites in the Glowworm Cave from which long-term data is obtained (after de Freitas and Schmekal, 2003: 76)
The experimental field data is collected specifically for this research through detailed monitoring experiments carried out inside the cave over a period of approximately nine months from March 2012 through December 2012. Each experiment is conducted over a period of three days (72 hours). Weekends are selected for data collection to ensure reasonable visitor numbers in order to capture occasions when the cave might be at its most vulnerable.
For each experiment, 10-minute interval measurements of air temperature and relative humidity are made using Kestrel 4500 Weather Tracker Meters at eight sites in the cave. In addition to the CO2 data collected by the AMES at the Organ Loft and Cathedral sites, Vaisala GMP222 sensors are installed at two other sites to measure air CO2 concentrations in the lower reaches of the cave, namely the Banquet Chamber and the Jetty sites. Air temperature and humidity are also recorded outside the Upper Entrance. Sensor locations are given in (Figure 5).
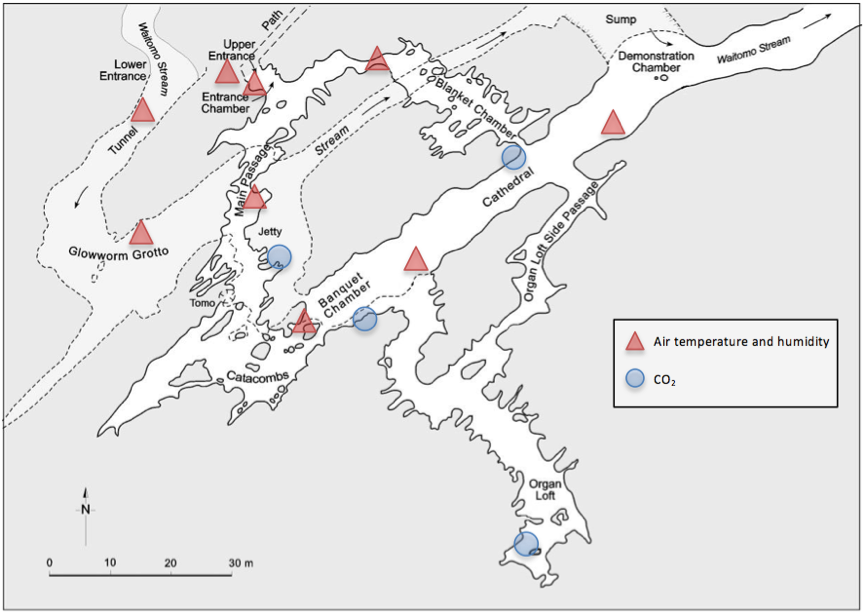
Figure 5: Sensor locations for experiments carried out in the Glowworm Cave (after de Freitas and Schmekal, 2003: 76).
Air samples of cave microclimatic variables, CO2 concentrations, and airflow are simultaneously recorded so that the potential drying impact of air temperature and the moisture gradient (as influenced from external atmospheric conditions) may be determined. To provide a simulated thermal gradient profile, which is created by external air entering and moving through the cave, measurements are taken from the cave entrances towards the interior of the cave. With all elements of cave management, environmental conditions, and other miscellaneous circumstances known and accounted for, these experimental field data serve to explain and validate statistical findings of the long term data archive through the use of detailed time series data and cave profile plots.
Td is a variable used as an indicator for airflow rate and direction. A simple equation for this, as used by de Freitas et al. (1982), is given as:
Td = To – Tc [°C] (1)
where To is air temperature outside the cave and Tc is air temperature in the cave interior, for which the Banquet Chamber is used as an indicator site. Based on (1), occurrences of downflow return positive Td values and upflow as negative Td values, using criteria established by de Freitas et al. (1982).
Air moisture content can be determined through specific humidity (q) of cave air. This is a function of vapour pressure (e) and may be defined as the mass of water per unit mass of moist air, or the relative density of water vapour in the air. As given by Grace (1983), q is expressed as:
q = (εe) / (P – e(1 –ε))/1000 [g kg-1] (2)
where P is the atmospheric pressure and εis the ratio of the molecular mass of water to the apparent molecular mass of dry air (= 0.6189 kg). q is used to gauge the level of cave air dryness in this study.
Day-to-day change in peak CO2 levels is not only a function of visitor numbers (Vn) and ventilation (Td). It would also depend on the CO2 level at the start of the visitor day, which may be elevated due to poor flushing during the previous night. Overnight recovery of CO2 is dependent on Td being sufficiently large to flush the cave (de Freitas and Banbury, 1999). To account for this, CO2 concentration at the start of the visitor day (CO2(0900)) is included as an independent variable in the regressions.
With the above in mind, both archived long-term and detailed experimental field data are used. The archived dataset is examined via a combination of stepwise regression and correlation analysis to determine the extent to which peak daily cave air CO2 levels can be attributed to Td, Vn, and CO2(0900). Calculations are carried out using Microsoft Excel 2011 and IBM SPSS Statistics 19. Case studies are undertaken using short-term data over the visitor day to reveal detailed real-time relationships to assess aspects of ventilation and ventilation control of the cave in more detail.
Results
The statistical procedure of multiple linear regression analysis allows prediction of values of a dependent variable from several other related independent variables. In this analysis, multiple linear stepwise regression models are used. Each independent variable is entered in sequence and its value assessed. If adding the variable contributes significantly to an increased explained variance (R2), it is retained. All other variables in the model are then re-tested as another is added to ensure each is still contributing to the success of the model. Removal of variables that no longer significantly contribute is based on the F-level.
Independent predictor variables that showed good correlation and will be used are: mean visitor numbers inside the cave throughout the visitor day (Vn), CO2 level recorded at the start of the visitor day (0900 hours) (CO2(0900)), maximum upflow Td (negative values) for the visitor day (Tdᵐᵃˣ↑), and maximum downflow Td (positive values) for the visitor day (Tdᵐᵃˣ↓). To ensure that only data from times of normal operating conditions were used, flood days and days of cave closure were removed from the analysis. Table 1 summarises the descriptive statistics and analysis results. F-level to enter is 0.05 and F-level to remove is 0.10. The Pearson’s correlation coefficients (R) show that all independent variables are positively and significantly correlated with CO2ᵐᵃˣ under upflow conditions (combined R = 0.87). For downflow conditions, Vn and CO2(0900) are positively and significantly correlated (combined R = 0.69) while no correlation exists with Tdᵐᵃˣ↓ (R = 0.04). Under upflow conditions at the Cathedral, and with all three predictors included, linear stepwise regression modelling produced a strong significant positive relationship, where R2 = 0.75, F (3, 1,236) = 1,245.4, and p < 0.001. Similarly, except under downflow conditions, the model produced a moderate significant positive relationship, where R2 = 0.47, F (3, 1,135) = 228.7, and p < 0.001.
Overall, the values for Pearson’s correlation coefficient (R) and the coefficient of determination (R2) show that visitor numbers are the main determinant of daily peak CO2 levels during both downflow and upflow conditions, accounting for up to 52% of the variation in CO2. The regression model for downflow conditions displays a much weaker relationship between variables than that for upflow conditions. The Tdᵐᵃˣ independent variables do not significantly contribute to either model, accounting for only 3 per cent and 9 per cent of CO2ᵐᵃˣ variability during upflow and downflow conditions respectively. This is likely due to a large range of CO2ᵐᵃˣ levels at Tdᵐᵃˣ values for visitor days.
These results suggest that, firstly, there is a causal relationship between daily peak CO2 values and the number of visitors in the cave, and secondly, that the driving force of ventilation may be nullified by cave-door management. Management of the cave is, therefore, successful in terms of airflow regulation based on the fact that it has overcome the effects of ventilation.
Cathedral CO2max for upflow conditions |
|
|
||||||||||||||||||||||||||||||||||||||||||||||||||||||||||||||||||||||||||||||||||||||||||||||||||||||||||||||||||||||||||||||||||||||||||||||||||||||||||||||||||||||||||||||||||||||||||||||||||||||||||||||||||||||||||||||||||||||||||||||||||||||||||||||||||||||||||||||||||||||||||||||||||||||||||||||||||||||||||||||||||||||||||||||||||||||||||||||||||||||||||||||||||||||||||||||||||||||||||||||||||||||||||||||||||||||||||||||||||||||||||||||||||||||||||||||||||||||||||||
Correlation coefficients (R) for variables |
|
|
||||||||||||||||||||||||||||||||||||||||||||||||||||||||||||||||||||||||||||||||||||||||||||||||||||||||||||||||||||||||||||||||||||||||||||||||||||||||||||||||||||||||||||||||||||||||||||||||||||||||||||||||||||||||||||||||||||||||||||||||||||||||||||||||||||||||||||||||||||||||||||||||||||||||||||||||||||||||||||||||||||||||||||||||||||||||||||||||||||||||||||||||||||||||||||||||||||||||||||||||||||||||||||||||||||||||||||||||||||||||||||||||||||||||||||||||||||||||||||
|
Vn |
CO2(0900) |
Tdmax↑ |
CO2max |
|
|||||||||||||||||||||||||||||||||||||||||||||||||||||||||||||||||||||||||||||||||||||||||||||||||||||||||||||||||||||||||||||||||||||||||||||||||||||||||||||||||||||||||||||||||||||||||||||||||||||||||||||||||||||||||||||||||||||||||||||||||||||||||||||||||||||||||||||||||||||||||||||||||||||||||||||||||||||||||||||||||||||||||||||||||||||||||||||||||||||||||||||||||||||||||||||||||||||||||||||||||||||||||||||||||||||||||||||||||||||||||||||||||||||||||||||||||||||||||
Vn |
1.000 |
0.304 |
0.345 |
0.719 |
|
|||||||||||||||||||||||||||||||||||||||||||||||||||||||||||||||||||||||||||||||||||||||||||||||||||||||||||||||||||||||||||||||||||||||||||||||||||||||||||||||||||||||||||||||||||||||||||||||||||||||||||||||||||||||||||||||||||||||||||||||||||||||||||||||||||||||||||||||||||||||||||||||||||||||||||||||||||||||||||||||||||||||||||||||||||||||||||||||||||||||||||||||||||||||||||||||||||||||||||||||||||||||||||||||||||||||||||||||||||||||||||||||||||||||||||||||||||||||||
CO2(0900) |
|
1.000 |
0.571 |
0.649 |
|
|||||||||||||||||||||||||||||||||||||||||||||||||||||||||||||||||||||||||||||||||||||||||||||||||||||||||||||||||||||||||||||||||||||||||||||||||||||||||||||||||||||||||||||||||||||||||||||||||||||||||||||||||||||||||||||||||||||||||||||||||||||||||||||||||||||||||||||||||||||||||||||||||||||||||||||||||||||||||||||||||||||||||||||||||||||||||||||||||||||||||||||||||||||||||||||||||||||||||||||||||||||||||||||||||||||||||||||||||||||||||||||||||||||||||||||||||||||||||
Tdmax↑ |
|
|
1.000 |
0.610 |
|
|||||||||||||||||||||||||||||||||||||||||||||||||||||||||||||||||||||||||||||||||||||||||||||||||||||||||||||||||||||||||||||||||||||||||||||||||||||||||||||||||||||||||||||||||||||||||||||||||||||||||||||||||||||||||||||||||||||||||||||||||||||||||||||||||||||||||||||||||||||||||||||||||||||||||||||||||||||||||||||||||||||||||||||||||||||||||||||||||||||||||||||||||||||||||||||||||||||||||||||||||||||||||||||||||||||||||||||||||||||||||||||||||||||||||||||||||||||||||
CO2max |
|
|
|
1.000 |
|
|||||||||||||||||||||||||||||||||||||||||||||||||||||||||||||||||||||||||||||||||||||||||||||||||||||||||||||||||||||||||||||||||||||||||||||||||||||||||||||||||||||||||||||||||||||||||||||||||||||||||||||||||||||||||||||||||||||||||||||||||||||||||||||||||||||||||||||||||||||||||||||||||||||||||||||||||||||||||||||||||||||||||||||||||||||||||||||||||||||||||||||||||||||||||||||||||||||||||||||||||||||||||||||||||||||||||||||||||||||||||||||||||||||||||||||||||||||||||
Model Step |
Variable entered |
R |
R2 |
R2 Change |
Std. Error of the Estimate |
p-value |
||||||||||||||||||||||||||||||||||||||||||||||||||||||||||||||||||||||||||||||||||||||||||||||||||||||||||||||||||||||||||||||||||||||||||||||||||||||||||||||||||||||||||||||||||||||||||||||||||||||||||||||||||||||||||||||||||||||||||||||||||||||||||||||||||||||||||||||||||||||||||||||||||||||||||||||||||||||||||||||||||||||||||||||||||||||||||||||||||||||||||||||||||||||||||||||||||||||||||||||||||||||||||||||||||||||||||||||||||||||||||||||||||||||||||||||||||||||||
1 |
Vn |
0.719 |
0.516 |
0.516 |
197.9 |
<0.001 |
||||||||||||||||||||||||||||||||||||||||||||||||||||||||||||||||||||||||||||||||||||||||||||||||||||||||||||||||||||||||||||||||||||||||||||||||||||||||||||||||||||||||||||||||||||||||||||||||||||||||||||||||||||||||||||||||||||||||||||||||||||||||||||||||||||||||||||||||||||||||||||||||||||||||||||||||||||||||||||||||||||||||||||||||||||||||||||||||||||||||||||||||||||||||||||||||||||||||||||||||||||||||||||||||||||||||||||||||||||||||||||||||||||||||||||||||||||||||
2 |
CO2(0900) |
0.849 |
0.204 |
0.204 |
150.6 |
<0.001 |
||||||||||||||||||||||||||||||||||||||||||||||||||||||||||||||||||||||||||||||||||||||||||||||||||||||||||||||||||||||||||||||||||||||||||||||||||||||||||||||||||||||||||||||||||||||||||||||||||||||||||||||||||||||||||||||||||||||||||||||||||||||||||||||||||||||||||||||||||||||||||||||||||||||||||||||||||||||||||||||||||||||||||||||||||||||||||||||||||||||||||||||||||||||||||||||||||||||||||||||||||||||||||||||||||||||||||||||||||||||||||||||||||||||||||||||||||||||||
3 |
Tdmax↑ |
0.867 |
0.031 |
0.031 |
142.0 |
<0.001 |
||||||||||||||||||||||||||||||||||||||||||||||||||||||||||||||||||||||||||||||||||||||||||||||||||||||||||||||||||||||||||||||||||||||||||||||||||||||||||||||||||||||||||||||||||||||||||||||||||||||||||||||||||||||||||||||||||||||||||||||||||||||||||||||||||||||||||||||||||||||||||||||||||||||||||||||||||||||||||||||||||||||||||||||||||||||||||||||||||||||||||||||||||||||||||||||||||||||||||||||||||||||||||||||||||||||||||||||||||||||||||||||||||||||||||||||||||||||||
Cathedral CO2max for downflow conditions |
|
|
||||||||||||||||||||||||||||||||||||||||||||||||||||||||||||||||||||||||||||||||||||||||||||||||||||||||||||||||||||||||||||||||||||||||||||||||||||||||||||||||||||||||||||||||||||||||||||||||||||||||||||||||||||||||||||||||||||||||||||||||||||||||||||||||||||||||||||||||||||||||||||||||||||||||||||||||||||||||||||||||||||||||||||||||||||||||||||||||||||||||||||||||||||||||||||||||||||||||||||||||||||||||||||||||||||||||||||||||||||||||||||||||||||||||||||||||||||||||||||
Correlation coefficients (R) for variables |
|
|
|
|||||||||||||||||||||||||||||||||||||||||||||||||||||||||||||||||||||||||||||||||||||||||||||||||||||||||||||||||||||||||||||||||||||||||||||||||||||||||||||||||||||||||||||||||||||||||||||||||||||||||||||||||||||||||||||||||||||||||||||||||||||||||||||||||||||||||||||||||||||||||||||||||||||||||||||||||||||||||||||||||||||||||||||||||||||||||||||||||||||||||||||||||||||||||||||||||||||||||||||||||||||||||||||||||||||||||||||||||||||||||||||||||||||||||||||||||||||||||||
|
Vn |
CO2(0900) |
Tdmax↓ |
CO2max |
|
|
||||||||||||||||||||||||||||||||||||||||||||||||||||||||||||||||||||||||||||||||||||||||||||||||||||||||||||||||||||||||||||||||||||||||||||||||||||||||||||||||||||||||||||||||||||||||||||||||||||||||||||||||||||||||||||||||||||||||||||||||||||||||||||||||||||||||||||||||||||||||||||||||||||||||||||||||||||||||||||||||||||||||||||||||||||||||||||||||||||||||||||||||||||||||||||||||||||||||||||||||||||||||||||||||||||||||||||||||||||||||||||||||||||||||||||||||||||||||
Vn |
1.000 |
0.241 |
0.422 |
0.523 |
|
|
||||||||||||||||||||||||||||||||||||||||||||||||||||||||||||||||||||||||||||||||||||||||||||||||||||||||||||||||||||||||||||||||||||||||||||||||||||||||||||||||||||||||||||||||||||||||||||||||||||||||||||||||||||||||||||||||||||||||||||||||||||||||||||||||||||||||||||||||||||||||||||||||||||||||||||||||||||||||||||||||||||||||||||||||||||||||||||||||||||||||||||||||||||||||||||||||||||||||||||||||||||||||||||||||||||||||||||||||||||||||||||||||||||||||||||||||||||||||
CO2(0900) |
|
1.000 |
0.317 |
0.450 |
|
|
||||||||||||||||||||||||||||||||||||||||||||||||||||||||||||||||||||||||||||||||||||||||||||||||||||||||||||||||||||||||||||||||||||||||||||||||||||||||||||||||||||||||||||||||||||||||||||||||||||||||||||||||||||||||||||||||||||||||||||||||||||||||||||||||||||||||||||||||||||||||||||||||||||||||||||||||||||||||||||||||||||||||||||||||||||||||||||||||||||||||||||||||||||||||||||||||||||||||||||||||||||||||||||||||||||||||||||||||||||||||||||||||||||||||||||||||||||||||
Tdmax↓ |
|
|
1.000 |
0.035 |
|
|
||||||||||||||||||||||||||||||||||||||||||||||||||||||||||||||||||||||||||||||||||||||||||||||||||||||||||||||||||||||||||||||||||||||||||||||||||||||||||||||||||||||||||||||||||||||||||||||||||||||||||||||||||||||||||||||||||||||||||||||||||||||||||||||||||||||||||||||||||||||||||||||||||||||||||||||||||||||||||||||||||||||||||||||||||||||||||||||||||||||||||||||||||||||||||||||||||||||||||||||||||||||||||||||||||||||||||||||||||||||||||||||||||||||||||||||||||||||||
CO2max |
|
|
|
1.000 |
|
|
||||||||||||||||||||||||||||||||||||||||||||||||||||||||||||||||||||||||||||||||||||||||||||||||||||||||||||||||||||||||||||||||||||||||||||||||||||||||||||||||||||||||||||||||||||||||||||||||||||||||||||||||||||||||||||||||||||||||||||||||||||||||||||||||||||||||||||||||||||||||||||||||||||||||||||||||||||||||||||||||||||||||||||||||||||||||||||||||||||||||||||||||||||||||||||||||||||||||||||||||||||||||||||||||||||||||||||||||||||||||||||||||||||||||||||||||||||||||
Model Step |
Variable entered |
R |
R2 |
R2 Change |
Std. Error of the Estimate |
p-value |
||||||||||||||||||||||||||||||||||||||||||||||||||||||||||||||||||||||||||||||||||||||||||||||||||||||||||||||||||||||||||||||||||||||||||||||||||||||||||||||||||||||||||||||||||||||||||||||||||||||||||||||||||||||||||||||||||||||||||||||||||||||||||||||||||||||||||||||||||||||||||||||||||||||||||||||||||||||||||||||||||||||||||||||||||||||||||||||||||||||||||||||||||||||||||||||||||||||||||||||||||||||||||||||||||||||||||||||||||||||||||||||||||||||||||||||||||||||||
1 |
Vn |
0.523 |
0.274 |
0.274 |
214.4 |
<0.001 |
||||||||||||||||||||||||||||||||||||||||||||||||||||||||||||||||||||||||||||||||||||||||||||||||||||||||||||||||||||||||||||||||||||||||||||||||||||||||||||||||||||||||||||||||||||||||||||||||||||||||||||||||||||||||||||||||||||||||||||||||||||||||||||||||||||||||||||||||||||||||||||||||||||||||||||||||||||||||||||||||||||||||||||||||||||||||||||||||||||||||||||||||||||||||||||||||||||||||||||||||||||||||||||||||||||||||||||||||||||||||||||||||||||||||||||||||||||||||
2 |
CO2(0900) |
0.621 |
0.385 |
0.111 |
197.4 |
<0.001 |
||||||||||||||||||||||||||||||||||||||||||||||||||||||||||||||||||||||||||||||||||||||||||||||||||||||||||||||||||||||||||||||||||||||||||||||||||||||||||||||||||||||||||||||||||||||||||||||||||||||||||||||||||||||||||||||||||||||||||||||||||||||||||||||||||||||||||||||||||||||||||||||||||||||||||||||||||||||||||||||||||||||||||||||||||||||||||||||||||||||||||||||||||||||||||||||||||||||||||||||||||||||||||||||||||||||||||||||||||||||||||||||||||||||||||||||||||||||||
3 |
Tdmax↓ |
0.687 |
0.473 |
0.088 |
183.0 |
<0.001 |
Table 1: Summary statistics, correlations, and results from stepwise regression analysis for CO2ᵐᵃˣ at the Cathedral site. R is Pearson’s correlation coefficient and R2 is the coefficient of determination. F-level to enter is 0.05 and F-level to remove is 0.10.
In light of the above multiple stepwise regression results, a ‘working’ model can now be applied to forecast CO2ᵐᵃˣ using a raw score model to compute predicted concentrations at the Cathedral for Tdᵐᵃˣ↑ (CO2ᵐᵃˣ↑) and Tdᵐᵃˣ↓ (CO2ᵐᵃˣ↓). A predictive tool such as this could be useful to cave operators so that high levels of CO2 can be anticipated and prevented. Linear models are developed and calibrated using data from 2008 to 2011 and are validated using data from 2012. Unstandardized coefficients from all three predictors are included in the models given as,
Cathedral CO2ᵐᵃˣ↑ = (Vn × 5.564) + (CO2(0900) × 0.614) + (Tdᵐᵃˣ↑ × 18.091) + 550.0444
and
Cathedral CO2ᵐᵃˣ↓ = (Vn × 4.877) + (CO2(0900) × 0.645) + (Tdᵐᵃˣ↓ × -25.989) + 607.603
The computed CO2ᵐᵃˣ values help to predict conditions which may lead to CO2 exceedances. It is also important to consider that, although the values estimated by the models are expected to be better than if CO2ᵐᵃˣ values were predicted by being simply assigned to the mean for the dataset, some values will be predicted more accurately than others. However, while the Cathedral CO2ᵐᵃˣ↑ and CO2ᵐᵃˣ↓ models, for example, account for 75 per cent and 47 per cent of the variance respectively, predicted CO2ᵐᵃˣ levels for each accordingly have an average error of 25 per cent and 53 per cent – not trivial amounts given the scale of CO2ᵐᵃˣ. In addition to the reliance of a given value on the fit of the model, each specific CO2ᵐᵃˣ estimate also depends upon the varying predictive power of individual variables involved. Therefore, when compared to observed values (Figure 6 and Figure 8), the calibration periods appear to successfully predict seasonal trends (most pronounced for Tdᵐᵃˣ↑, given in Figure 6) and middle range CO2ᵐᵃˣ values with reasonable accuracy, but become less accurate at extreme high-end and low-end concentrations. The daily maximums for the validation period (2012) are almost always under-predicted, although the typical seasonal trend that is evident in the observed data is maintained by the model. The variation between observed and predicted CO2ᵐᵃˣ for calibration and validation is given in Figure 7 and Figure 9. Improvements to the fit of each model are seen for the 2012 validation periods, where R2 increases by 3 per cent for Tdᵐᵃˣ↑ and by 4 per cent for Tdᵐᵃˣ↓. This is likely to be due to the reduced variation of CO2 maximums, as well as absence of very high values that the model is prone to under-predicting.
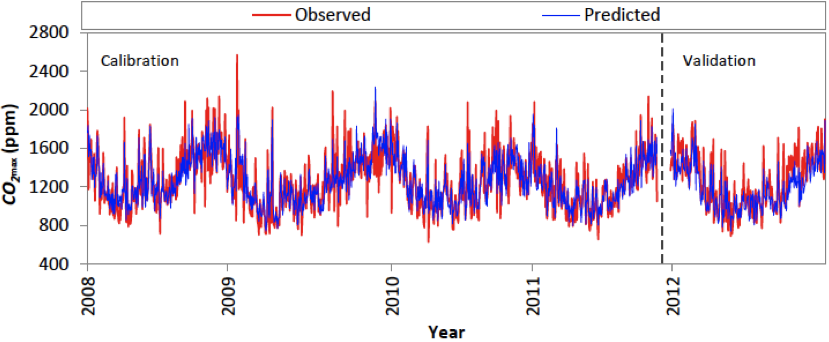
Figure 6: Observed CO2ᵐᵃˣ and predicted (modelled) CO2ᵐᵃˣ for Tdᵐᵃˣ↑ at the Cathedral based on computed estimates obtained using a raw score model. Calibration period is 2008-2011 and validation period is 2012.
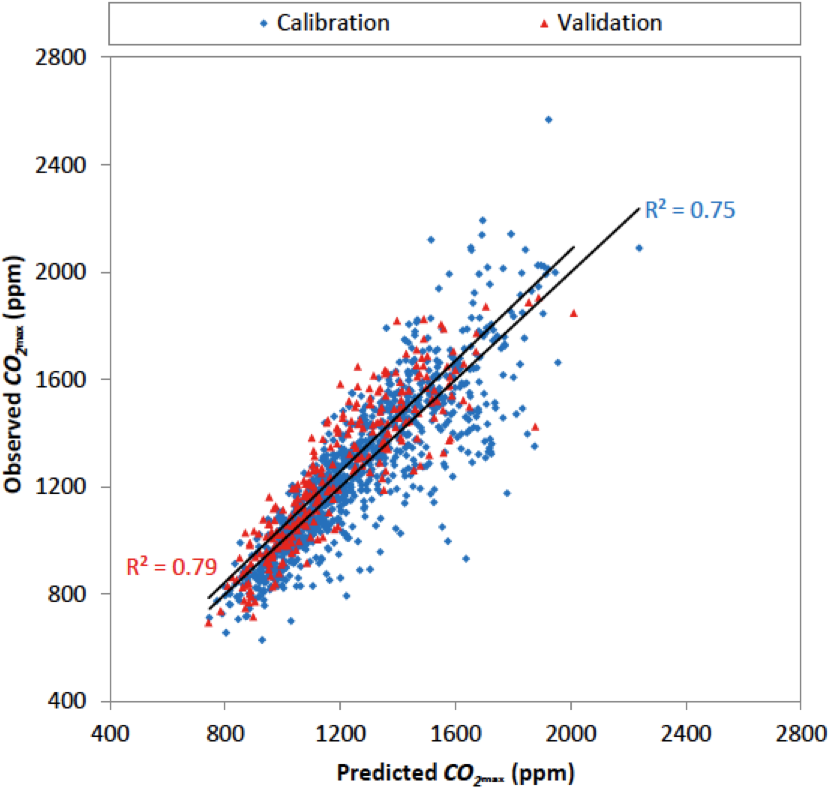
Figure 7: Observed CO2ᵐᵃˣ and predicted (modelled) CO2ᵐᵃˣ for Tdᵐᵃˣ↑ (CO2ᵐᵃˣ↑) at the Cathedral for the calibration (2008-2011) and validation (2012) datasets. Regression equation is: CO2ᵐᵃˣ↑ = (Vn × 5.564) + (CO2(0900) × 0.614) + (Tdᵐᵃˣ↑ × 18.091) + 550.0444
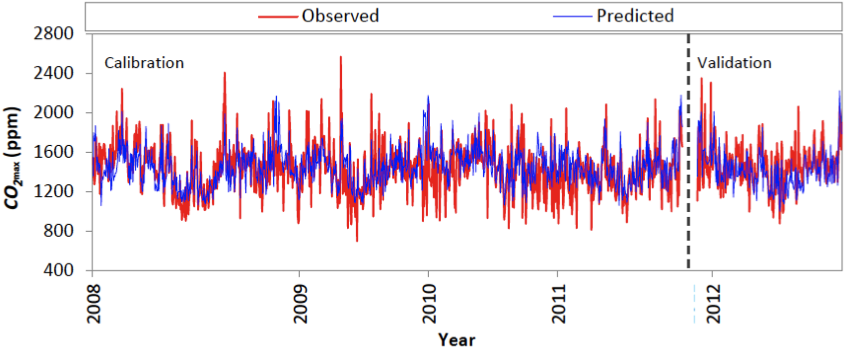
Figure 8: Observed CO2ᵐᵃˣ and predicted (modelled) CO2ᵐᵃˣ for Tdᵐᵃˣ↓ at the Cathedral based on computed estimates obtained using a raw score model. Calibration period is 2008-2011 and validation period is 2012.
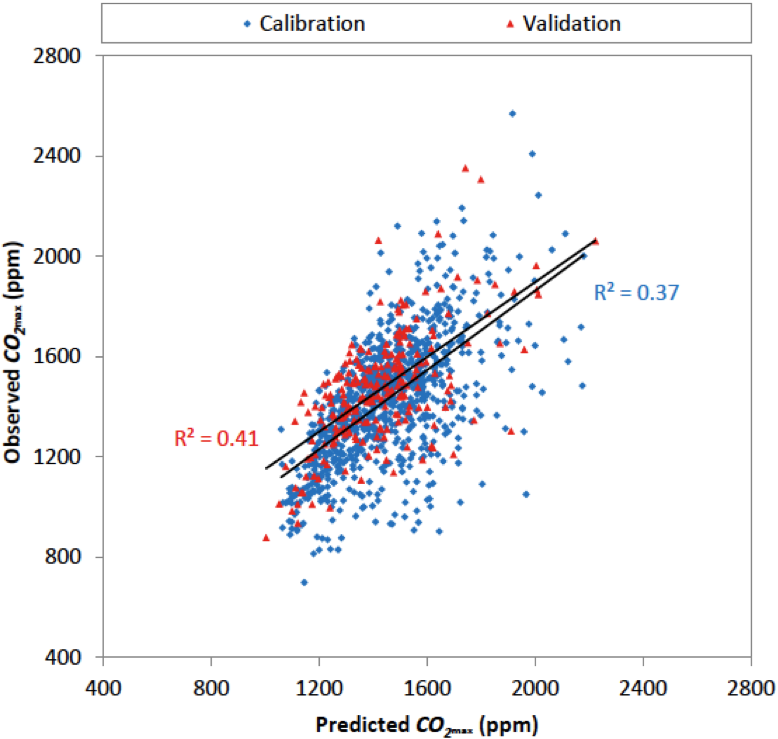
Figure 9: Observed CO2ᵐᵃˣ and predicted (modelled) CO2ᵐᵃˣ for Tdᵐᵃˣ↓ (CO2ᵐᵃˣ↓) at the Cathedral for the calibration (2008-2011) and validation (2012) datasets. Regression equation is: CO2ᵐᵃˣ↓ = (Vn × 4.877) + (CO2(0900) × 0.645) + (Tdᵐᵃˣ↓ × -25.989) + 607.603
To further explore the nature of the relationship during down-flow conditions, a case study was conducted using half-hourly data from 0900 to 1800 h on January 2, 2010. This was a relatively warm summer’s day during the peak of the cave’s tourist season. Data for CO2 in the Organ Loft are included to assess ventilation. Visitors to the cave are not routinely taken to the Organ Loft as CO2 builds up rapidly in this cul-de-sac passage. The results in Figure 10 show that, despite the absence of visitors to the Organ Loft the build-up of CO2 is greatest. This is because the Cathedral is better ventilated as it lies in the main airflow route through the cave. Consequently, CO2 concentrations are generally lower in the Cathedral, with peak values almost one third lower. The results in Figure 10 also show the lag between the Organ Loft and Cathedral for peak CO2 values, a phenomenon first discovered by de Freitas and Banbury (1999).
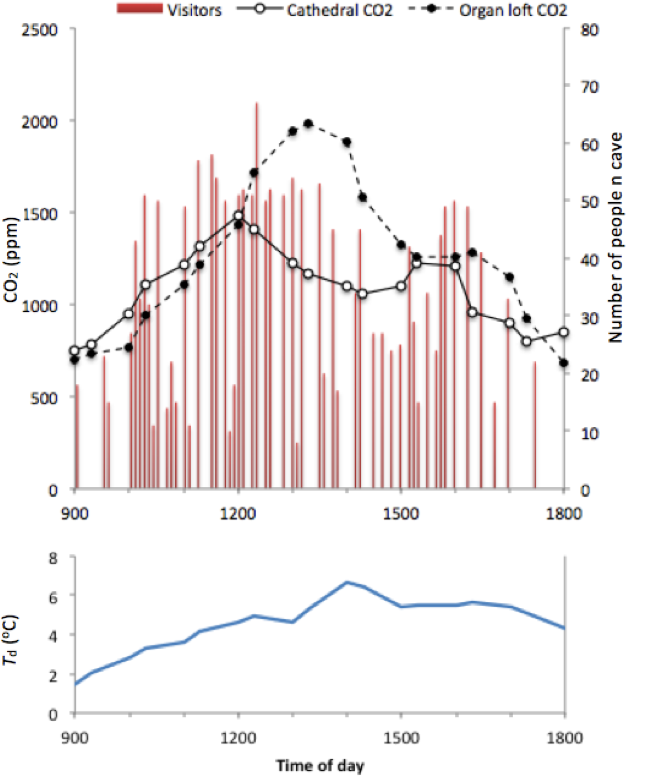
Figure 10: Effect of tour groups on CO2 levels in the Cathedral and Organ Loft during one visitor day on January 2nd 2010. Each vertical line represents a visitor tour. CO2 data are half-hourly. Concentrations of CO2 ranged from an initial 700pmm at both sites to 1,500 ppm later in the day in the Cathedral and 2,000 ppm in the Organ Loft.
The below results are based on the ‘experimental field data’ collected from experiments conducted over 72 hours in the Glowworm Cave in 2012. These data are used to demonstrate the impact of visitor use and cave management on CO2 levels. Data is explored through detailed time series and cave profile plots. For the experiment conducted over the period 20 to 22 October 2012, a series of circumstances came together to make the cave vulnerable to high CO2 levels. This experiment extends Labour Day weekend. This is an annual national public holiday during which many people make short trips to tourist destinations such as Waitomo. Hence many larger than usual half-hourly tour groups of 40 or more people visited the cave during the peak periods of the first two recording days (Saturday and Sunday). The maximum number of people inside the cave on Day 1 was 188 at 1230 hours, and this increased to 204 people at 1530 hours on Day 2 (Figure 11a). On Day 3 the cave was closed to visitors. For the duration of the experiment Td was near zero, so ventilation was minimal even with the door open. To compound the situation, 67 mm of rain fell over 24 hours (Figure 11d), which by mid afternoon on the second day saw the cave at near ‘pipe-full’ conditions at the lower entrance. In addition, runoff water flowing into the stream is likely to have picked up organic matter that would have been washed into the cave, releasing additional CO2 into the cave environment.
The relatively large number of visitors on the first two days is a key factor in the ensuing events leading to an exceedance of the cave air CO2 2,400 ppm limit (Figure 11a). On Day 1, before any rainfall, conditions in the cave were generally as per usual. Many people passed through the cave, which saw CO2 rise until the cave door was left open for two hours (Figure 11b). A reasonable rate of upflow, with a mean Td of around -4.0 ºC, allowed the cave to recover from raised CO2 levels (Figure 11c). For the remainder of the experiment ventilation rates are small throughout entire diurnal cycles and hence the effectiveness of cave door management as a tool for reducing high CO2 concentrations was significantly reduced.
By 1230 hours on Day 2 the water was declared too high for boat tours to operate, and visitors were made to retrace their steps at the conclusion of tours to exit through the Upper Entrance. At this point, and with the stream water level continuing to rise (resulting in a reduced area for air exchange to occur at the Lower Entrance), cave air CO2 responded by rapidly increasing. Unlike for Day 1, the action of opening the cave door at 1100 hours had no real mitigating effect. The continuing rise in cave air CO2 levels ceased quite promptly once the final large tour group of 33 people exited the cave at 1700 hours. This demonstrates that although cave air CO2 could not be reduced through opening the cave door, the alternative management tool of restricting the tour groups is an effective one. During the evening of Day 2 cave air CO2 lowered at all sites to be maintained at about 1,900 ppm. Overnight, Td is minimal and the stream at the Lower Entrance is at near ‘pipe-full’ flow so only minor CO2 recovery occurred.
According to the real-time CO2 measurements available to supervisors over this period, the cave was managed effectively because cave air CO2 levels at the Organ Loft and Cathedral indicator sites (both located at the upper-level) peaked under the 2,400 ppm limit. However, measurements recorded at the Jetty and Banquet Chamber lower-level sites (not taken into account by management) tell a different story, showing much higher peak CO2 levels and both exceeding the 2,400 ppm limit. This would suggest the cave was not managed effectively.
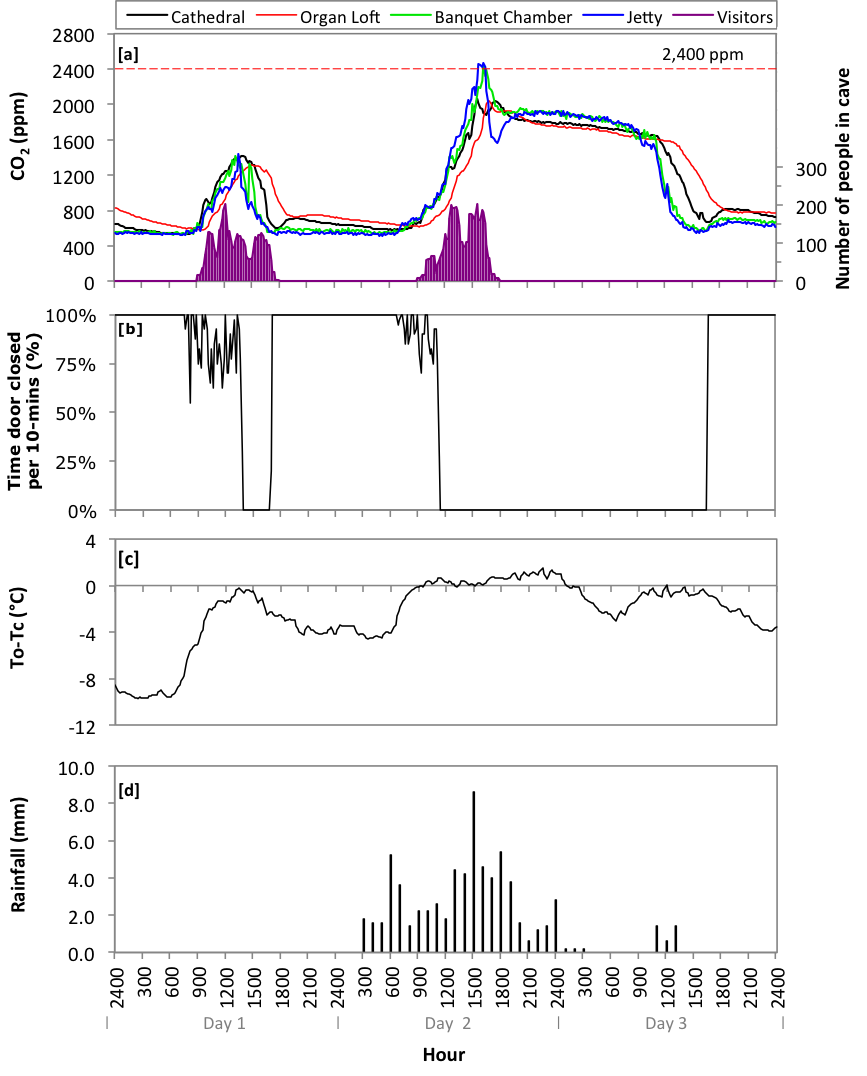
Figure 11: 10-minute experimental field data for days 20-22 October 2012. Panel [a] is cave air CO2 alongside total visitors inside the cave, [b] is percentage time the door is closed per 10-minutes, [c] is the cave-to-outside-air temperature gradient, and [d] is rainfall.
Using the experimental field data, this study examines the processes involved in longitudinal spatial distribution of Td, q, and relative humidity (rh) within the cave under upflow and downflow conditions. The key concepts from observations can be briefly summarised into four main points to give context for these results: 1) movement of external air into the cave has a modifying effect on cave air temperature (Tc) and rh through advection of heat and moisture from outside the cave; 2) the air moving through the cave is also modified by exchange of heat and moisture with the cave surfaces; 3) while air entering cave entrances has the thermal and moisture properties of outside atmosphere, it quickly adopts to the characteristics of the cave atmosphere; and 4) through advection, this air is modified as it moves deeper into the cave towards a heat and moisture equilibrium with the cave environment.
During upflow conditions, air temperatures increase and the amplitude of air temperature variation tends to decrease with distance into the cave from the Lower Entrance. Spatial trends for q inside the cave also mimic those of air temperature, whereby q increases (increasing moisture holding capacity of cave air) and amplitude of variation decreases with distance from the cave entrance. An exception occurs at the Entrance Chamber site where Tc and q levels are lower than those at the Main Passage, which is positioned deeper into the cave. This is likely due to its close proximity to the Upper Entrance where the cave door allows cooler outside air into the cave. Even small amounts of cool air infiltration can generate a near-entrance cold zone. This offsets the heating effect of the warmer cave air and passage surfaces.
A schematic of the thermal regime for an upflow profile is given in Figure 12. The spatial distribution of q, Td, and rh during strong upflow conditions for the Glowworm Cave is presented in the form of a graphical cave profile plot given in Figure 13. The profile can be explained with reference to physical processes of heat and moisture transfer. The results show air moving up through the cave from the Lower Entrance, taking actual values for all sites measured at 0730 hours on 27 July 2012. This is when the minimum Td value from within the experimental data (or strongest upflow) was recorded. In theory, this is the point at which the cave is most vulnerable to evaporative cooling and drying.
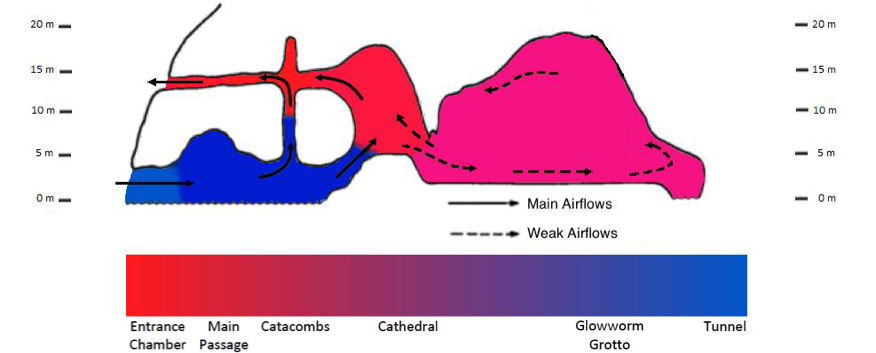
Figure 12: Schematic of cave airflow regime: upflow profile. Colours used are relative to other cave sections rather than indicative of actual temperatures (after de Freitas et al., 1982).
Measurements of Td, q, and rh are given in Figure 13, which illustrates the spatial distribution for very strong upflow in the morning, when Td is within the range of -11.0 ºC to -14.0 ºC, external rh is high at 85 per cent, and q of outside air (qo) at 3.6 g kg-1 is lower than q of cave air (qc) at 9.5 g kg-1 (mean of all cave sites).
Due to low outside air temperatures, the increase in cave air temperature upon reaching the Main Passage is as large as 14 ºC (Figure 13). There is, however, a small decrease in Tc and q between the Main Passage and Entrance Chamber. This is caused by infiltration of cooler outside air near the Upper Entrance, forming a cold zone that mixes with the warmer, moister cave air. Saturation of cave air is the result and condensation will occur on the cool surfaces within the Entrance Chamber.
The spatial distribution of air temperature within the cave is shown in Figure 13. The tendency is for Tc to increase, and thus Td to decrease with distance from the Lower Entrance moving towards upper-level through deep-cave sites. The spatial distribution of q and rh in the cave profile increases with distance from the Lower Entrance. This begins when the dryer outside air is advected through the Lower Entrance and into the cave. For example, in Figure 13, the cool outside air entering the cave through the Lower Entrance, on reaching the tunnel site less than 10 m away, has achieved an increase of 4.6 g kg-1 q and very quickly warmed to 11.5 ºC. The change in q once air is expelled from the cave at the Upper Entrance is 6.5 g kg-1.
At the same time and somewhat counter-intuitively, as incoming outside air is warmed, its evaporating potential is increased causing drying in the cave. This effect is most pronounced in the Lower Entrance zone (Figure 13). The large thermal gradient leads to energy transfer from the relatively warm and moist cave air and surfaces into the cooler outside air moving through the cave. The capacity of the air parcel to hold moisture increases as air temperature rises, while moving upwards through or deeper into the cave with increasing distance from the Lower Entrance. The constant heat and moisture exchange remains the case even when relative humidity is at, or close to, 100 per cent because the increasing temperature allows the vapour flux to continue. Thus it is possible that evaporation is occurring throughout the cave if a sufficient moisture supply is available. For example, in Figure 13 a 3 ºC rise in Tc between the Tunnel and Main Passage sites increases the moisture holding capacity of the air by 1.9 g kg-1.
It is reasonable to assume that there is sufficient moisture available at the stream-level Glowworm Grotto and Tunnel sites so that the air here maintains saturation. Between the Glowworm Grotto and Main Passage, which are dryer sections of the cave, there is evidence of evaporation; thus drying occurs since the vapour flux (moisture supply) is not sufficient to meet the evaporative demand. Because of this, the variability of q and rh between sites moving upwards is determined by the vapour content of outside air, net moisture advection, the vapour flux between the air and cave surfaces, and the distribution of moisture within the cave. In addition, the spatial distribution of Td in the cave is determined by external air temperature and q, the advection of heat from outside the cave, as well as sensible and latent heat flux between cave air and cave surfaces.
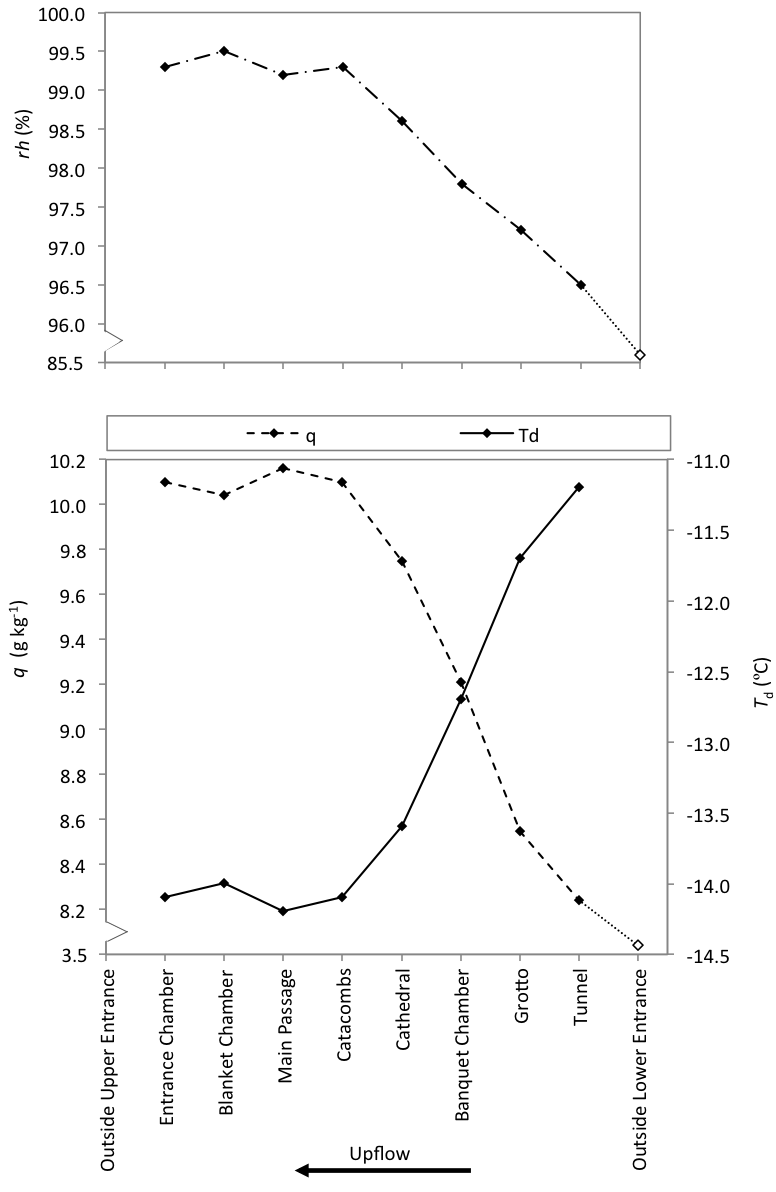
Figure 13: Cave q and Td profiles for upflow conditions, 0730 hours, 27 July 2012. Values for q are also given for air outside the Lower Entrance (dotted connector line).
Under downflow conditions, the amplitude of temperature variation tends to lessen with distance into the cave from the entrances, while temperatures decrease as warm air entering the cave cools. Spatial trends for qc are directly related to those of Tc, where qc increases and the amplitude of variation decreases with distance from the cave entrances. The variation of Tc between all other sites, moving deeper into the cave, is significantly smaller and generally less than 1.0 ºC.
A schematic of the thermal regime for the following downflow cave profiles is given in Figure 14. The spatial distribution of q, Td, and rh under downflow conditions in the cave is presented in the cave profile plot given in Figure 15. The profile used in the analysis show external air entering the cave via the Upper Entrance and moving downwards and out the Lower Entrance. The case taken occurs at 1500 hours on 14 December 2012. It typifies the distribution for when downflow Td is greatest.
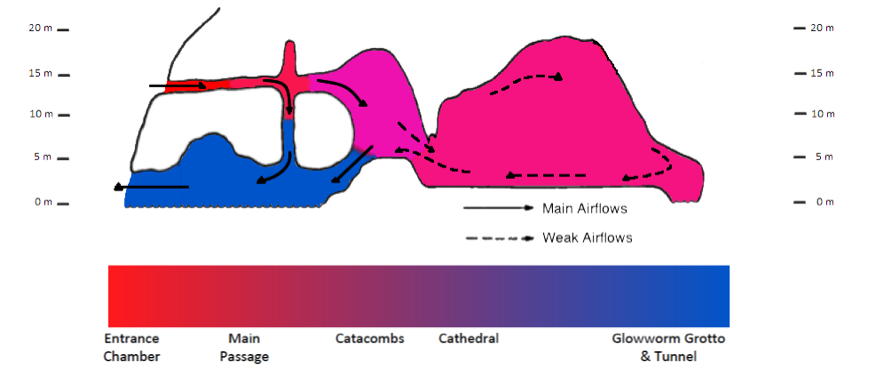
Figure 14: Schematic of cave airflow regime: downflow profile. Colours used are relative to other cave sections rather than indicative of actual temperatures (after de Freitas et al., 1982).
Illustrated in Figure 15 is the spatial distribution for strong downflow, where Td is within the range of 3.0 ºC to 6.0 ºC, external rh relatively high at 80 per cent, and qo (14.0 g kg-1) is higher than mean qc (10.9 g kg-1). As the outside air temperature is relatively high in the given profile, the reduction in air temperature between the Upper Entrance and the Banquet Chamber is large. This is as much as 8.2 ºC in Figure 15, where outside air temperature at the Upper Entrance is 23 ºC. A rise in Tc and q is then observed between the Banquet Chamber and Tunnel sites. Air temperature recorded at the Tunnel site (located near the Lower Entrance) is higher than might be expected. This is likely due to increased temperature of water flowing into the cave via the Waitomo Stream, which is typically the case during summer months wherein the warmer stream water emits heat into cave air to adjust the stream-level microclimate.
The spatial distribution pattern shown in Figure 15 is such that Tc decreases, and thus Td increases, with distance from the Upper Entrance. There is a decrease in q and increase in rh for both profiles with distance from the Upper Entrance. The process observed is warm, moist outside air moving through the Upper Entrance and into the cave, cooling, and losing its moisture holding capacity. The change in q once air reaches the Lower Entrance is 3.1 g kg-1, while the air temperature has lowered by 4.7 ºC. Compared to the relatively large initial change in q (5.0 g kg-1) observed in the strong upflow Td profile (Figure 13), a smaller initial change in q for air entering the cave (3.0 g kg-1) is observed in the downflow profile.
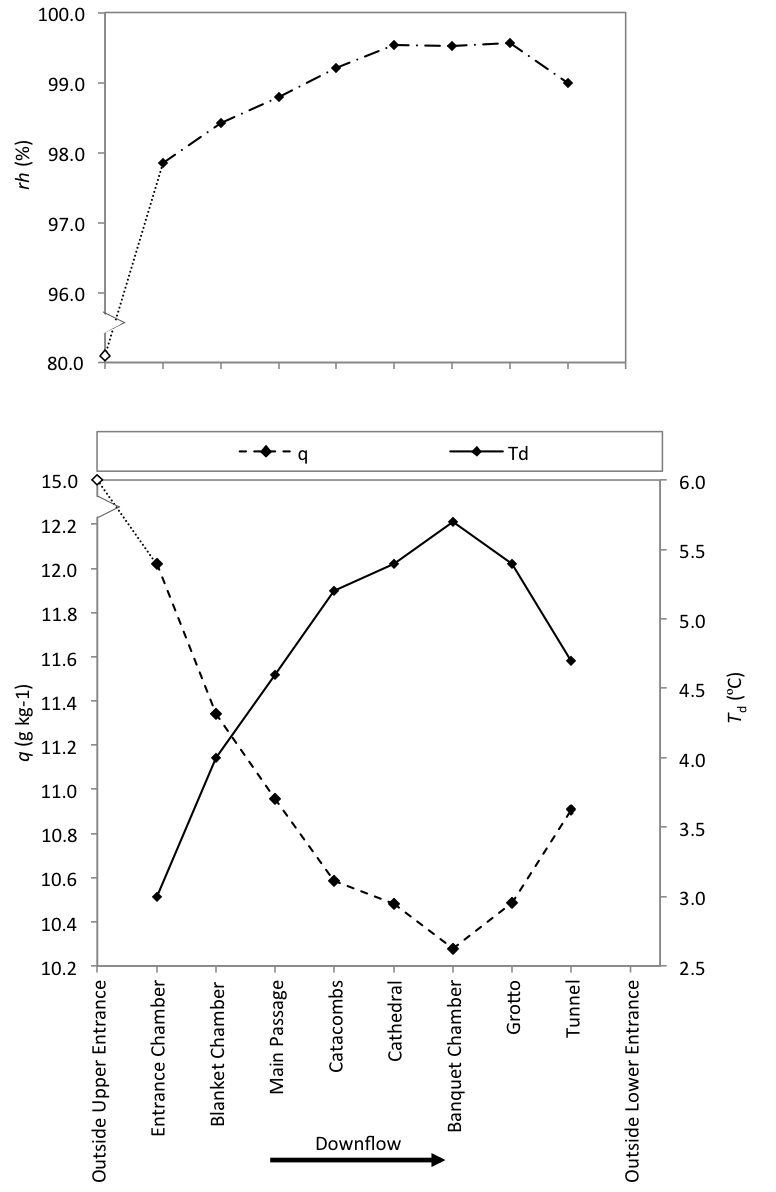
Figure 15: Cave q and Td profiles for downflow conditions, 1500 hours, 14 December 2012. Values for q are also given for air outside the Upper Entrance (dotted connector line).
Discussion and Conclusion
The results show that daily peak CO2 levels are largely insensitive to Td. This suggests that cave management through ventilation control is effective, especially during downflow conditions, but only when Td is sufficiently high to allow CO2 drainage. Downflow occurs when the outside air is warmer than the air in the cave. Typically, condensation will occur in the cave along with some warming, but the latter is small because ventilation rates are relatively low (de Freitas and Schmekal, 2003). Upflow occurs when conditions outside are relatively cool or cold and below mean cave air temperature. Cold weather causes cave drying if air exchange with the cave is facilitated. For this reason, cave management guidelines dictate that cave ventilation should be minimised by keeping the upper entrance of the cave sealed when upflow conditions exist, other than when visitors enter the cave. However, if visitor numbers are not reduced or carefully controlled, CO2 build-up will occur. The results of this research indicate this to be the case in that peak CO2 is more highly correlated with visitor numbers.
The examination of high-frequency time series data is revealing and the results support the findings of the regression analysis. There can be a considerable difference in daily peak CO2 levels for the different sections of the cave, with different degrees of spatial remoteness and ventilation efficiency appearing to have a significant effect on CO2 build-up and diffusion rates.
The main determinant of cave climate is air exchange with the outside atmosphere. Sites within the cave closest to the entrances have the greatest range in temperature and humidity. The amplitude of variation between sites decreases with increasing distance from entrances. Cave profile plots constructed from the experimental field data show that cave air moisture varies longitudinally and is determined by the:
- vapour content of outside air
- amount of moisture moving into or out of the cave via advection
- vapour flux between cave air and surfaces through condensation and evaporation; and
- distribution of moisture throughout the cave
The results show that there is a marked difference between the upper-level sites, which are dryer sections of the cave, and lower-level sites. In the case of the former, there is evidence of relatively high evaporation rates that cause drying because the moisture supply is not sufficient to meet the evaporative demand. Under downflow conditions, the warmer air entering the cave generally has higher moisture content than that of the cave, so moisture availability is not an issue and condensation is more likely to occur.
Key Recommendations
The research presented here paves the way for further long-term monitoring and research projects that investigate the impacts of visitors in sections of the Glowworm Cave outside those covered by the AEMS. By carrying out field experiments of longer durations, seasonal cave air temperature and humidity distribution can be understood more comprehensively. These projects should be designed so that they are able to contribute new information that could be used for management of the cave environment.
Ideally, current management guidelines that treat the cave as a whole should be adjusted to more ‘micro-management’ based guidelines that regard the Glowworm Cave as a series of caves or caverns within a cave. These caverns may then be managed separately and according to their individual microclimates, degrees of remoteness, ventilation rates, and morphological differences. This requires a set of baseline microclimate conditions and CO2 concentration thresholds and lag-times to be established for multiple parts of the cave.
To advance understanding of the spatial and temporal differences in CO2 concentrations in the cave, more CO2 monitoring stations in the cave are required. Potential monitoring sites could be at the Jetty and Banquet Chamber, where CO2 levels are shown in this work to reach higher peaks than those observed at the Cathedral and Organ Loft during periods of high visitation, flooding, and small cave-to-outside-air temperature difference. Installation of further wet and dry-bulb temperature probes throughout the cave is also desirable so that spatial variability of air temperature and humidity can be monitored in greater detail. However, the installation of at least one or two additional CO2 sensors should be given priority. Future studies that investigate the role of organic matter and soils on CO2 levels in the cave would advance knowledge and understanding of CO2 sources and rates of accumulation. Given this is a temperate cave system with vast areas of vegetation and soil input above the cave, this would be an ideal environment in which to carry out such a study.
References
de Freitas, C. R. (2010). The role and importance of cave microclimate in the sustainable use and management of show caves. Acta Carsologica, 39(3): 477-489.
de Freitas, C. R. and K. Banbury. (1999). Build up and diffusion of carbon dioxide in cave air in relation to visitor numbers at the Glowworm Cave, New Zealand. Cave management in Australasia XIII. Proceedings of the thirteenth Australasian conference on cave and karst management, Mount Gambier, South Australia, Australasian Cave and Karst Management Association, Carlton South, Victoria. pp 84-89.
de Freitas, C. R., R. N. Littlejohn, T. S. Clarkson and I. S. Kristament. (1982). Cave climate: assessment of airflow and ventilation. Journal of Climatology, 2(4): 383-397.
de Freitas C. R. and A. Schmekal. (2003). Condensation as a microclimate process: Measurement, numerical simulation and prediction in the Glowworm Cave, New Zealand. International Journal of Climatology, 23(5): 557-575.
Grace J. (1983). Plant-Atmosphere Relationships. Chapman Hall, London, 92 p.
O’Brien, B. J. and J. R. Watson. (1977). The paradox of cave management: to conserve or use. Proceedings of the Second Australian Conference on Cave Tourism and Management, Hobart. pp 97-104.