Dynamics of soil - vegetation systems on limestone and karst management
INTRODUCTION
Most textbook discussions of the limestone solution process tend to concentrate on chemical kinetics and on hydrology, especially those parts of the process occurring within active cave conduits. A notable exception is Trudgill's (1976) text on limestone geomorphology. Yet the veneer of soil on most limestones has a central role in karst processes, through its control in water infiltration and storage, by acting as a CO2 generator and through the role of soil buffering capacity in the solution process. Globally limestone soils support large populations and historically have been badly degraded by over-use: two examples are the denudation of Mediterranean karsts since 1500BC and the more recent "rocky desertification" of the classic Chinese towerkarsts by over-clearing and acid rain. (Huntoon, 1992). Today an enhanced understanding of the role of soils and vegetation is helping to limit the extent of land degradation on limestone areas.
SOIL AND VEGETATION IN THE KARST SOLUTION PROCESS
The roles of soil and vegetation in the solution process can be outlined using Figure 1. Healthy perennial vegetation acts to intercept rainfall and its dissolved gases, principally carbon dioxide (CO2). Atmospheric carbon dioxide is currently 0.034% of the total volume and is increasing at approximately 5% of its concentration per annum. To this can be added other aerosols such as sulphuric acid from industrial sources and often oxides of nitrogen formed in thunderstorms. The patchy cover of vegetation intercepts rain and protects the soil surface from rain and wind erosion. At the ground surface the infiltration of rainwater is aided by leaf litter and hollows which allow most water to percolate into the soil; the excess runs off to surface channels. The complex of decaying vegetation, soil fauna (including bacteria) and fungi in leaf litter provide a source of carbon dioxide and additional organic compunds such as humic and fulvic acids. In the root zone the respiration of up to 25% of the carbon dioxide taken up by plants occurs. This released gas may dissolve in percolating rainwater. Bacteria in topsoil also release copious quantities of the gas through their metabolism, and are the major producers. In acidic soils bacteria are disadvantaged: decomposition of organic matter is achieved by fungi which are much slower yet also yield carbon dioxide. These processes result in soil CO2 concentrations of between 0.1 and 3.5% in temperate regions, and up to 10% in the tropics. Much of this gas dissolves in soil water to give a weak acid which is carried down by gravity to the subsoil along with humic and other organic acids .
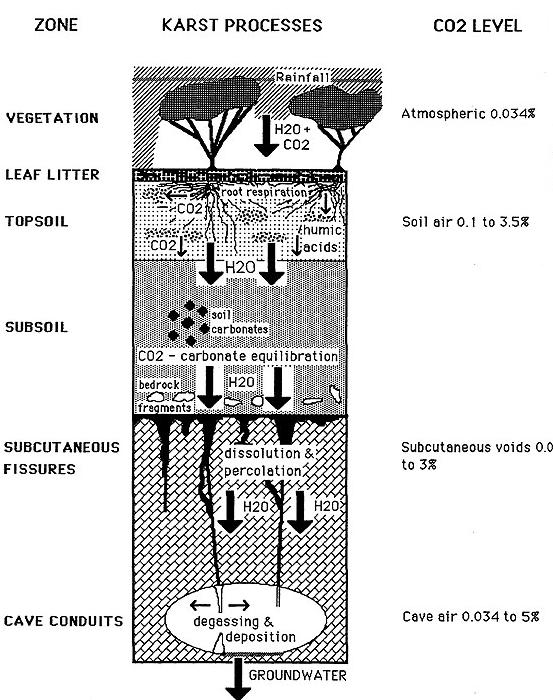
Figure 1: Schematic of the dynamics of the solution process in surface karst.
In the subsoil aeration is much less and bacterial action is reduced due to this and to lower organic matter content. The important process of carbon dioxide - calcium carbonate equilibration takes place in this zone. Carbon dioxide gas dissolves in water to give the weak carbonic acid, which dissociates to hydrogen and hydrogen carbonate ions. An increase in CO2 concentration in the soil will allow more hydrogen ions to be released into drainage water, acidifying the soil (lower soil pH). This greatly enhances the ability of the percolating water to dissolve calcium carbonate. Some or all of the weak acid may be neutralised by exchangeable calcium ions released from clays, from carbonate concretions or from bedrock fragments. Thus bedrock erosion is much reduced where the soil has a high exchangeable calcium content released from leaf litter, or where it contains a high proportion of bedrock fragments. Changes in soil pH may be reduced by the reserve, or buffering capacity, of hydrogen ions derived from organic acids released from the decomposition of clay-humus particles and organic matter. The chemical balance of the karst solution process is thus very dependent on organic influences and especially the maintenance of vegetation , leaf litter and a productive topsoil. Where native, calcium-loving vegetation is replaced by plants adapted to more acidic soils, then the soil may become acidified to bedrock and acelerated erosion of limestone occurs. This has been the case in Yorkshire where more frequent burning has encouraged the growth of heather at the expense of the natural grassland and woodland vegetation: there are now deep runnels formed in bedrock down which soil can be lost (Trudgill, 1976). A similar process has been observed in New Guinea where slash and burn cultivation on limestone is accompanied by greatly accelerated soil loss (Gillieson et al., 1986).
LIVING ON LIMESTONE: A BOTANICAL VIEWPOINT
There are a number of interactions between limestone soils and vegetation. The free vertical drainage of most limestone soils creates special conditions for evapotranspiration, gas exchange and root penetration. Large eucalypts act as water pumps, taking up 250-270L/day for each tree of river red gum. Tree roots can penetrate to depths of 30-50m in search of water, especially so in seasonally humid climates of northern Australia. Up to 25% of the carbon dioxide taken in by trees is released back into the soil by root respiration. Litter retention is of great importance for nutrient cycling, with most release by decomposition occurring within six months of litter fall for karri in W.A. The vegetation structure (especially projected foliage cover) is important for interception of rainwater, soil water infiltration and temperature control in soil and subcutaneous zones. This directly affects both the quantity and quality of water available as feed water for speleothem growth in underlying caves. The penetration of tree roots, and the release of complex organic acids and phenolic compounds by them, aids the enlargement of bedrock fissures in karst and ensures the high degree of secondary porosity characteristic of limestone terrains.
Native plants growing on limestone are frequently displaced by exotic species, creating management problems. Most plant species can be classified according to their life strategies - their life-forms, resistance to disturbance, rates of seed production and its viability. Highly competitive species such as blackberries grow rapidly, resist disturbance, bear copious viable seed and may inhibit the establishment and growth of other species near them by poisoning the soil (allelopathy). Stress-tolerating plants such as figs and eucalypts have adaptations to ensure adequate water supply (deep roots, high root-shoot ratios), to resist fire (resistant bark, lignotubers, and vegetative reproduction) and to exploit low levels of nutrients (root fungi associations, high root hydrogen ion production). Ruderal species, those that habitually colonise disturbed habitats such as roadsides, have tuberous energy stores, very effective seed dispersal, and easily pollinated flowers. Dandelions and St John's Wort are good examples of ruderal plants.
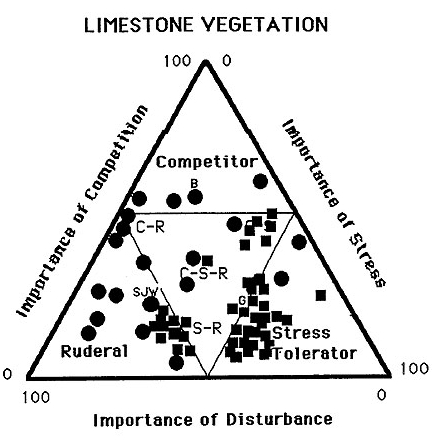
Figure 2: Stress -competition triangle for limestone vegetation. Data for Australian species (squares) from personal observations in NSW & ACT, European data (circles) from Grime (1979).
These functional plant groups can be defined and placed on a stress-competition triangle (Grime, 1979). In Australia stress-tolerating plants are generally more numerous on limestone soils (Figure 2) with some ruderals, but highly competitive species are rare. In part this explains why many karst areas of Australia have been colonised by competitive exotic species which naturally grow in limestone areas of Europe and North America. Those native species which are stress-tolerators are very susceptible to displacement by exotic species when the disturbance regime is altered radically. Their reproductive strategies may be adapted to infrequent disturbance and they may be unable to complete their reproductive cycle if repeatedly stressed. This may happen as a result of increased fire frequency, after earth moving operations or after accelerated erosion. In the case of fire, the transference of prescriptions derived for one area to another may not produce the same response in vegetation. There is some evidence suggest that native species are "plastic" in their response to fire disturbance, resprouting on some sites but dying at others (Williams et al, 1994). A change in the vegetation may therefore have major consequences for karst hydrology and the limestone solution process. This has been noted for the replacement of native woodland by exotic pines with higher water use and a tendency to acidify the soil, resulting in interruption of growth and even erosion of speleothems in caves below the introduced forest.
SOIL MANAGEMENT ON KARST
Erosion prediction and control on limestone soils follows the same provisions as most other soil types with some important exceptions. Water erosion by sheet and rill processes may be predicted at a point using the Universal Soil Loss Equation (USLE). This has been calibrated for some Australian soils from long-term erosion plot studies (Rosewell & Edwards, 1988). Annual soil loss in tonnes/hectare (A) is a function of the rainfall intensity or erosivity (R), the soil erodibility (K), the slope angle and length (L,S), vegetation cover (C) and cultivation practices (P):
A = R x K x L x S x C x P
The best treatment of this subject for local conditions is given by the SOILOSS package of Rosewell & Edwards (1988) or the Victorian soil conservation manual (Garvin et al, 1979). Since soil erosion is a function of ground slope and slope length, any factors locally increasing the slope angle will result in more erosion. On non-karstic terrain, the slope angle and length are functions of the slope distance to the nearest drainage line. On limestone the intimate connections between the surface and the underground cave conduit network lead to locally steeper hydraulic gradients which enhance erosive processes. There is also greater potential for vertical abstraction of material down joints and fissures by sinkhole collapse, gullying, or soil stripping. The preservation of adequate vegetation cover and of soil structure (reducing erodibility) assumes greater importance on limestone than elsewhere in mitigating this effect.
Caesium-137 has been used extensively as a tool to measure rates and patterns of soil redistribution. Fallout of this radionuclide is derived from atmospheric testing of atomic weapons during and after the 1950s, with the concentrations of 137Cs reaching levels detectable today in 1958 within Australia. After reaching the soil surface 137Cs has been shown to be irreversibly bound to soil particles, especially clays. Its fate and redistribution within the landscape thereafter only occurs by soil transport processes (Campbell et al, 1986). Its properties as a unique and discrete label of surface soil make it ideal as a tracer to test comparative rates of erosion, in which measurements of areal concentration of 137Cs in erosion or deposition sites are compared to the known total or 'reference' fallout at that location (Wallbrink & Murray, 1993). Increases in this isotope compared to the 'reference' value indicate areas of soil accretion and areas of decrease represent zones of erosion. These proportional differences may be converted to quantitative loss by a variety of models (Walling and Quine, 1990; Gillieson et al, 1994 and in press). These measurements allow us to make estimates of erosion integrated over a long time and thus free of the biases of short-term sampling which may miss major events in the landscape.
The literature of soil science is extensive and often complex. Under the widely used U.S.D.A. Soil Taxonomy a very large number (70) of soil properties must be measured before a soil can be classified and its behaviour predicted. This is also true for soil classifications based on soil formation or genesis, such as Great Soil Groups. Fortunately in Australia we use a simpler factual key for the classification of soils (Northcote, 1979) in which a few key properties are measured in the field. These are the soil profile form, the texture of the visible layers, their pH and colour. From these much of the behaviour of soils can be inferred:
- the profile form tells us about soil drainage, rooting ability of trees and aeration;
- soil texture tells us about the water holding ability and nutrient retention;
- pH tells us about nutrient availability and the acidity of soil water;
- colour tells us about organic matter content, clay minerals and precipitates.
To these should be added total organic matter content, soil water infiltration capacity and penetrometer resistance, a measure of compaction. These are all useful attributes of limestone soils which can be measured in the field and provide a good basis from which to predict soil behaviour, especially susceptibility to erosion and suitability for plant growth. They should be part of the basic toolkit for karst managers.
GREEN SOLUTIONS FOR LIMESTONE SOILS
Nearly all of the karst solution process is moderated by factors operating close to the surface of the karst, in the soil and in the subcutaneous zone. Surface vegetation regulates the flow of water into the karst through interception, the control of litter and roots on soil infiltration, and the biogenic production of carbon dioxide in the root zone. The metabolic uptake of water by plants, especially trees, may regulate the quantity of water available to feed speleothems. Trees in particular are like large carbon dioxide pumps, releasing much of the atmospheric gas uptake through root respiration. Thus clearfelling on karst, or major changes consequent on plantation establishment, may radically change the flow and quality of water in the karst. Soil erosion in excess of the natural rates may infill streamsinks, dolines or joints. Changes to surface drainage resulting from contour banking, irrigation or river regulation may interrupt or drastically reduce the supply of karst water. The release of fertilisers, herbicides and insecticides from agricultural activities may compromise cave ecosystems beyond their capacity to recover. Water is the primary mechanism for the transferral of surface actions to become subsurface impacts.
For karst areas, the concept of total catchment management becomes more vital than in many other lithologies. This involves the coordinated management and utilisation of physical resources of land, water and vegetation within the boundaries of a catchment to ensure sustainable use and to minimise land degradation. No government agency, private company or individual should be exempt from this. Proper environmental management on karst terrains rests on a base of public acceptance that clear linkages exist between surface and underground systems, and that these linkages are of fundamental importance to karst system function.
Defining the contributing catchment of a cave system may be difficult if not impossible in some cases. A minimalist approach would be to define the catchment as the area of limestone outcrop. This neglects the possibility that the limestone is continuous though not outcropping in a given terrain, or that surrounding non-karstic rocks are contributing significant quantities of water by surface or subsurface flow. In many cases a thick mantle of sediment overlies the limestone and directly feeds cave systems. This is especially true in areas which were formerly glaciated or which have been subject to repeated mass movements over geologic time. Subterranean breaches of surface drainage divides are often the norm rather than the exception, and the exact conditions for the activation of cave conduits may depend on storm events or antecedent rainfall. Thus the definition of a karst catchment is imprecise and must have a dynamic boundary to take account of extreme events. This is best achieved by constructing buffer zones around limestone massifs, in which any change to land use must be preceded by investigations of the drainage network and its dynamics using repeated dye-tracing experiments. Under these circumstances:
"One well-designed tracer test, properly done, and correctly interpreted, is worth 1000 expert opinions or 100 computer simulations of groundwater flow" (Jim Quinlan, pers. comm.).
It is important that clear objectives for soil management on karst be established before any work takes place. This necessitates extensive consultation between industry, government agencies and community interest groups. A primary objective has to be the removal of any sources of pollution, both surface and underground. This may involve sealing of landfills, excavation and removal of contaminated sediments, bioremediation using bacteria or plants, flushing of contaminated water or sediments from caves, or even excavation and removal of contaminants from caves. This is a costly process, and often a portion of the cost will have to be borne by those government agencies responsible for environmental management. This is especially so when the source of pollution is either very old or is community based e.g. sewage pollution or municipal garbage dumps. Erosion mitigation using surface stabilisation techniques, contouring and drainage control is a secondary objective which has to be approached armed with the best practicable knowledge of the underlying karst system. There are numerous examples of geotechnical solutions in karst which were conceived in haste and are now repented at leisure. Another important objective is the degree to which the original landscape will be simulated, and the final land use to be permitted. In England quarry rehabilitation has involved simulation of the natural geomorphic features of the karst, and revegetation for aesthetic and habitat values. The former can be achieved as the final stage in extraction using controlled blasts to create buttress and gully topography, and a variety of regolith textures (Gagen & Gunn, 1987). Such techniques may be inappropriate where biological conservation issues are important.
There are a number of basic principles for effective karst soil management and rehabilitation of degraded sites:
- Restore the hydrology of the site. It is crucial to have a high degree of secondary porosity to allow the flow of percolation water and groundwater recharge. This may involve ripping or removal of compacted soil or sediments, construction of drainage control banks, and removal of sediment from infilled dolines or joints.
- Control active soil erosion and sediment entry to the karst system. This may involve construction of contour banks, revegetation, filtration of drainage water at streamsinks, and stabilisation of steep slopes prone to mass movement.
- Get the soil biology working again. Invertebrates such as earthworms, ants and termites are effective at breaking down leaf litter, bioturbating the regolith, improving soil texture, increasing the quantity and availability of nutrients, and providing a food source for higher animals.
- Establish a stable vegetation cover, preferably of perennial plants. Permanent vegetation is effective at controlling erosion by water or wind, provides a source of slow-release nutrients, and is aesthetically pleasing.
- Monitor progress above and below ground. The success of rehabilitation and compliance with community standards can only be gauged by repeated sampling of karst waters. The sampling should be event based, so as to account for increased transfer of sediments and solutes during rainstorms. Ideally monitoring should be continuous using dataloggers and probes. Relationships between karst water chemistry and suspended load can be developed with simple parameters such as conductivity, pH and turbidity.
- Leave the site alone unless things go wrong. There is a great temptation to interfere with the rehabilitation when processes are slow, especially if political pressure is constant. Revegetation should ideally be only formally assessed after a minimum of two years, when sufficient establishment and growth has occurred. Clearly the site should be regularly examined for excessive sediment transport, and remedial action taken if necessary. For many karst areas, especially those where climate limits biological processes, rehabilitation time may be measured in decades.
REFERENCES
Campbell, B.L., Elliot, G.L. and Loughran, R.J.(1986): Measurement of soil erosion from fallout Caesium-137. Search 17: 148-149.
Gagen, P. and Gunn, J., 1987, A geomorphological approach to restoration blasting in limestone quarries. In Beck, B. F. and W. L. Wilson, Karst Hydrogeology: Engineering and Environmental Applications. Rotterdam: A. A. Balkema, 457-61.
Garvin, M.R. , Knight, M.R. & Richmond, T.J. (1979): Guidelines for minimising soil erosion and sedimentation from construction sites in Victoria, TC-13, Melbourne, Soil Conservation Authority, 279pp.
Gillieson, D., Oldfield, F. and Krawiecki, A. (1986): Records of Prehistoric Soil Erosion from Rockshelter Sites in Papua New Guinea, Mountain Research and Development, 6(4):315-324.
Gillieson, D., Cochrane, A. and Murray, A., (1994): Surface hydrology and soil erosion in an arid karst: the Nullarbor Plain, Australia. Environmental Geology 23: 141-147.
Gillieson, D., Wallbrink P., Murray, A. and Cochrane, A. (in press) Estimation of wind erosion using the radionuclide caesium-137 on the Nullarbor Plain karst, Australia. Zeitschrift fur Geomorphologie.
Grime, J. P. (1979): Plant strategies and vegetation processes, Chichester, Wiley.
Huntoon, P. (1992): Chairman Mao's Great Leap Forward and the deforestation ecological disaster in the south China karst belt, In Quinlan, J. & Stanley, A. (eds.) Conference on Hydrogeology, Ecology, Monitoring and Management of Ground Water in Karst Terranes, 3rd, Nashville, Tenn, Proceedings, National Well Water Assoc., Dublin, Ohio, pp 149-158.
Northcote, K. H., 1979 , A Factual Key for the Recognition of Australian Soils. (4th.ed) Adelaide, Rellim Technical Publications.
Rosewell, C.J. & Edwards, K. (1988): SOILOSS: a program to assist in the selection of management practices to reduce erosion, Soil Conservation Service of NSW Technical Handbook #11, Sydney, 71pp plus disk.
Trudgill, S.T. (1976): The erosion of limestone under soil and the long-term stability of soil-vegetation systems on limestone, Earth Surface Processes and Landforms 1: 31-41.
Trudgill, S.T. (1985): Limestone Geomorphology, London, Longmans.
Wallbrink, P.J. & Murray, A.S. (1993): Use of fallout radionuclides as indicators of erosion processes, Hydrological processes 7: 297-304.
Walling, D.E. and Quine, T.A.(1990): Calibration of Caesium -137 measurements to provide quantitative erosion rate data. Land Degradation and Rehabilitation. 2: 161-175.
Williams, J.E., Whelan, R.J. & Gill, A.M. (1994): Fire and environmental heterogeneity in southern temperate forest ecosystems: implications for management, Aust. J. Botany 42: 125-137.